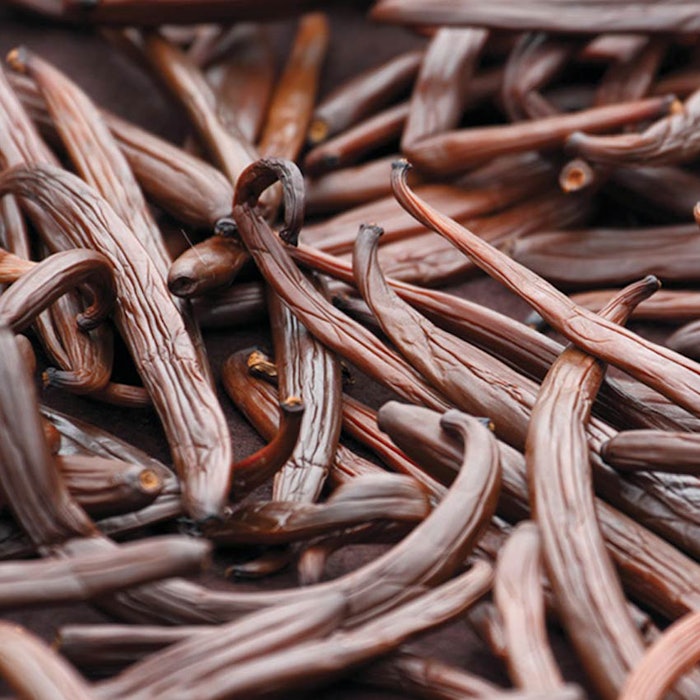
The cultivation and processing of many natural raw materials conducted in tropical or semi-tropical climates employ conditions that have not materially changed over the last 50 years.
Log in to view the full article
Introduction
The cultivation and processing of many natural raw materials conducted in tropical or semi-tropical climates employ conditions that have not materially changed over the last 50 years.
Our understanding of the molecular biology and biochemistry of plant tissues, especially the ripening and senescence processes, have developed extensively over the last 10-15 years. Newer ways of handling plant materials using milder processing have supplemented our capability to manipulate these systems. However, the exploitation of this understanding and these technologies in terms of improved natural materials processing has not been extensively implemented.
On the basis of this developing knowledge we have highlighted two key natural raw materials—tea and vanilla—and considered both the common and inherent differences these materials share regarding biochemistry, chemistry and processing. By taking a multidisciplinary approach, it may be possible to utilize information from these individual tissues to mutual benefit.
Tea Processing
Both green and black teas are manufactured from the young leaves of Camellia sinensis L. of the genus Camellia and the family Theaceae. By a spectrum of processing protocols—from mild to more robust—families of teas including white through green and oolong to black are produced. Fresh cut tea leaves contain between 75-80% moisture and a unique family of polyphenolic catechins whose concentration in the fresh tealeaf range from 13-25% of the dry weight. Four monomeric catechins comprise this group namely (-)-epicatechin (EC), (-)-epicatechin-3-O-gallate (ECg), (-)-epigallocatechin (EGC) and (-)-epigallocatechin-3-O-gallate (EGCg). The structures of (-)-epicatechin (EC) and (-)-epigallocatechin (EGC) are shown in F-1
Other constituents of note in the young leaves include amino acids, caffeine, the carotenoids and chlorophyll.
Tea processing includes, in its most protracted operation, the stages of withering and maceration, fermentation then finally firing and drying.
Oxidation reactions are responsible for the browning of tea leaves as well as the production of taste and aroma compounds in the finished products. Depending on the type of tea, oxidation is almost totally inhibited in white and green teas; or deliberately initiated, controlled then stopped which is seen in oolong and black tea a
Most of the oxidation processes involve the catechins and the oxido-reductase enzymes polyphenol oxidase (PPO) and peroxidase (POD) along with their respective hydrogen acceptors. Cell damage in the tea leaf initiates the interaction between molecular oxygen, the polyphenols in the cell vacuole, PODs in the cell peroxisome and cytoplasmic PPO.
In the same oxidative environment, chlorophylls are degraded to pheophytin and pheophorbide pigments, which contribute to the black/brown color of dry oxidized tealeaves. Lipids, amino acids and carotenoids are transformed to produce tea aroma compounds.
The process of withering involves placing the leaves in large open holding vessels in the sun or in a cool airy room for sufficient time to achieve about 20-25% moisture loss. This stage is followed by mechanical tissue damage by maceration or rolling or tumbling the tea leaves. Maceration is typically used to create CTC (cut, tear, curl) or other broken-leaf teas. Due to extensive cellular disruption, it is the most rapid route to initiate polyphenol oxidation and is achieved using a rotorvane or a CTC machine. Oxidation begins when the integrity of the cell walls within the tea leaves are compromised. Rolling is a mild process resulting in a much slower and gentler oxidation and is usually conducted using a rolling table or by hand. Tumbling is an even milder route to initiate oxidation and is achieved using large cylinders in which the leaves are tumbled.
Control of oxidation in the fermentation stage is managed by introducing warm, moist, air. The extent to which oxidation is allowed to occur has a significant effect on the finished tea. Oxidation occurs optimally at temperatures between 27o-30oC and relative humidity of 98%. Oxidation is virtually inhibited at 60o-66oC by heating the leaves and denaturing the oxido-reductase enzymes.
Firing (drying) is achieved by heating the leaves to approximately 66oC.
The most common firing methods are pan firing, steaming, heat tumbling or baking. Alternative processes include sun drying, microwaving or hot water blanching. The drying process realizes a final leaf moisture content of 2-3%.
If oxidation is totally prevented, tea leaves retain their green color and appearance and the catechins remain largely intact (white and green teas).
When a semi-oxidized tea is produced, partial conversion of catechins to theaflavins and the latter to thearubigins occurs, resulting in a slight browning in the leaves and a yellower liquor (oolong tea).
When fully oxidized black tea leaves exhibit an aroma and taste profile totally distinguishable from un-oxidized finished tea. Drying is conducted to achieve reduced moisture content for shelf stability and for flavor enhancement. For the latter roasting may be employed to generate toasty and burnt notes as well as a darker appearance.
Tea Taste Compounds
The key biochemical reaction relevant to the taste of tea occurs mainly during fermentation and is characterized by the oxidation of catechins by PPO with molecular oxygen as the hydrogen acceptor. The initial products from the reaction are a family of dimeric theaflavins (F-2). Theaflavins possess a characteristic benzotropolone moiety, which is produced by condensation between a catechol-type B-ring of EC and a pyrogallol-type B-ring of EGC.
Oolong tea
The theaflavins are reddish in color and contribute briskness and astringent character to tea. In vitro oxidation of a mixture of EC and EGC with polyphenol oxidase suggested that the enzyme preferentially oxidizes EC to EC-quinone. This electron deficient EC-quinone then reacts with the electron-rich EGC B-ring. Subsequent oxidation and decarboxylation afforded theaflavins. These compounds are not final products and are oxidized further as EGC was consumed faster than EC during theaflavin formation since oxidative coupling between two molecules of EGC readily occurs. When EGC is exhausted in the reaction mixture, the EC-quinone begins to oxidize theaflavin via the electron withdrawing action of the EC-quinone from the benzotropolone ring. This transformation of theaflavins leading to thearubigins is catalyzed by POD with hydrogen peroxide as the hydrogen acceptor. The thearubigens are red in color and contribute body to tea [2,3]. Several oxidation products of the theaflavins are known; the major one being theanaphthoquinone [4]. In black tea fermentation, coexisting substances may react with theaflavin quinones or theanaphthoquinone to form further complex polyphenols. The theaflavins and thearubigins, in combination, are the principal contributors to taste, mouth-feel and color of fermented teas.
Vanilla Taste Compounds
Traditional vanilla bean curing is characterized by the activation of hydrolytic and oxido-reductase enzymes. The ripe, green beans, like the young tea leaf contain mainly mono-phenols as the β-D-glucosides account for 12-15% of the dry weight of the vanilla pod. An endogenous β-glucosidase hydrolyzes the phenyl-β-D-glucosides to the free phenols among which the mono-phenol vanillin is the major compound generated. These phenols are then oxidized principally by PPO/molecular oxygen and/or POD/hydrogen peroxide to dimeric and polymeric “brown” pigments. A number of novel phenol oxidation products from cured vanilla beans were recently isolated and structurally determined. Some of these compounds have interesting taste and velvety mouth-feel attributes [5].
The above authors identified six compounds previously not reported in cured vanilla beans namely 5-(4-hydroxybenzyl) vanillin, 4-(4-hydroxybenzyl)-2-methoxyphenol, 4-hydroxy-3-(4- hydroxy-3-methoxybenzyl)-5-methoxybenzaldehyde, (1-O-vanilloyl)-(6-O-feruloyl)-ß-D-glucopyranoside, americanin A and 4’,6’-dihydroxy-3’,5-dimethoxy-[1,1’-biphenyl]-3- carboxaldehyde (F-4)
Sensory studies revealed human recognition thresholds for these compounds with velvety mouth-coating sensation between 1.0 and 5.0 μmol/kg in water. Quantitative analysis of the compounds in cured beans from different geographical origins as well as in non-cured beans revealed that, with the exception of americanin A, all the other taste compounds were absent in the green bean and found only after curing.
Concentration and taste threshold data for a number of these velvety mouth-coating compounds were determined. Highest concentration/taste threshold values, in the commercial Madagascan vanilla cured extracts evaluated, in parenthesis, were found for (1-O-vanilloyl)-(6-O-feruloyl)-β-D-glucopyranoside (234), di-vanillin (281) and 4’,6’-dihydroxy- 3‘,5’-dimethoxy-(1,1’-biphenyl)-3-carboxaldehyde (266). Similar data was obtained for cured Vanilla planifolia beans of Papua New Guinea origin. It is probable that these compounds contributed significantly to the taste/mouth-coating character of cured vanilla beans.
New Compounds Linked to Vanillin
Of the new compounds identified (1-O-vanilloyl)-(6-O-feruloyl)-β-D-glucopyranoside would seem to be linked to the known vanillin precursor, vanillin-O-β-D-glucopyranoside commonly known as gluco-vanillin. One of the other compounds was a di-phenol whilst three others were di-phenylmethanes. Di-vanillin, a known component of cured vanilla beans, was also identified. The routes of formation of these dimeric compounds may involve oxidation, via radical intermediates, mediated by POD/hydrogen peroxide and/or PPO/molecular oxygen. In the case of the di-phenol compounds, it is established that vanillin in the presence of horseradish peroxidase/hydrogen peroxide rapidly dimerises to di-vanillin [6]. The pathway to the di-phenylmethanes has not been defined though radical coupling of appropriate phenols, such as vanillin, vanillyl alcohol, guaiacol and other suitable phenols catalyzed by POD/hydrogen peroxide and/or PPO/molecular oxygen, which could be the route of formation. Rathore and Kochi (1995) described the synthesis of di-phenylmethanes via dealkylative coupling of benzylic ethers in the presence of the stable cation radical oxidizing agent Orange CRET [7].
These oxidation/browning reactions in vanilla beans were shown to be dependent on the presence of molecular oxygen. Under conditions where oxygen was limited or excluded, browning of vanilla beans does not occur even over a 10 day fermentation period at 60oC. Introduction of air into vanilla beans incubated in an anaerobic or anoxic environment resulted, within 2-3 hours, in brown coloration of the green beans [8]. The browning of ripe, green, vanilla beans under these conditions is a molecular oxygen dependent reaction. It also appears that anoxic or anaerobic conditions have a sparing effect on vanillin loss relative to that observed under aerobic conditions[9]. Both PPO and POD are active enzymes in vanilla pods [10,11]. PPO utilizes molecular oxygen as the hydrogen acceptor whilst hydrogen peroxide performs the same function for POD. It would seem that browning during vanilla bean fermentation is dependent on PPO/molecular oxygen, unless POD is active but limited in hydrogen peroxide.
Purified monomeric PPO from ripe vanilla beans with a molecular weight of ca.34 kDaltons utilizes as primary substrates the o-diphenols catechol and 4-methylcatechol. The products from oxidation of these substrates are the corresponding ortho-quinones [12]. Other o-diphenol substrates present in plants include caffeic and chlorogenic acids. The dominant feature of the biochemistry of o-quinones is their ability to readily undergo redox reactions involving a semi-quinone radical intermediate following single electron reduction. Ortho-quinones are reactive electron deficient species and when generated in biological environments they can react rapidly, often by non-enzymatic pathways, to give more stable products. The driving force for this reactivity is their conversion to aromatic catechols.
It would seem that browning during vanilla bean fermentation is dependent on polyphenol oxidase/molecular oxygen, unless peroxidase is active but limited in hydrogen peroxide.
Catechol formation occurs by reduction, by addition of a nucleophile, or by tautomerism followed by nucleophilic addition. The new catechols formed can readily be re-oxidized to new o-quinones, which by further functionalization can achieve more complex structures. Reaction of o-quinones with electron rich nucleophiles, such as amino acids, result in intra- or inter-molecular addition. With amino acids, the reaction is primarily through the -amino groups, with the exception of lysine and cysteine, to give red or brown adducts. The products of these reactions are mono-substituted phenols that may be oxidized further to colored quinone derivatives [13]. Ortho-quinones can react further with quinones, phenols, peptides and proteins. The reactions of o-quinones with amino acids compete less successfully with the non-enzymatic polymerization of o-quinones. In this manner, labile o-quinones may react together to yield “melanoid” type pigments. The mechanism of polymerization is not well defined since the final, colored, melanin products are dependent on the nature of the reactants.
The Role of Molecular Oxygen
The best understood o-quinone polymerization pathway is that of the conversion of tyrosine to melanin. Tyrosine is first hydroxylated to L-3,4-dihydroxyphenylalanine (DOPA). The latter is then oxidized to dopaquinone; both reactions were catalyzed by the PPO enzyme cresolase/O2. Dopaquinone cyclizes to leuco-dopachrome which is then oxidized to give orange dopachrome. Following a series of further reactions, dopachrome then polymerizes to melanin [14]. In the context of browning in vanilla beans during the fermentation stage, it is well established that the mono-phenol vanillin is readily oxidized by POD/hydrogen peroxide, an enzyme active in vanilla beans, to yield di-vanillin 6.
It is likely therefore, that phenol oxidation in fermenting vanilla beans proceeds to dimeric and polymeric, brown pigments, as follows:
- PPO/molecular oxygen oxidation of o-diphenols to the corresponding o-quinones which by further oxidation can realize complex colored polymeric structures, as well as reaction of the o-quinones with nucleophiles, such as the –NH2 groups of μ-amino acids to yield colored addition products
- Mono-phenols such vanillin, in the presence of POD/hydrogen peroxide, react to produce C-C bridged dimers
- Co-oxidation of phenols such as vanillin by the semi-quinone intermediates in the oxidation of o-diphenol by PPO/O2 [15].
The role of molecular oxygen in this browning scheme is evident. The PPO/O2 reaction is required for browning to occur. The primary role of this enzyme is to oxidize o-diphenols to the corresponding o-quinones. These reactive quinones can react with nucleophiles, such as µ-amino acids or polymerize to complex polyphenols. In addition, the o-quinones can oxidize monophenols, such as vanillin to dimeric di-phenols. The latter compounds may also be generated by reaction of the monophenol with POD/H2O2 (see F-5).
There are clear similarities and some differences between vanilla and tea taste compounds in terms of:
- Precursor molecules. In both cases phenolic and poly-phenolic respectively
- The enzymes responsible for the oxidation of the phenols. In both raw materials, the enzymes POD and PPO are the oxidation catalysts for the reactions, which take place principally during the fermentation phase following initial tissue damage.
- In tea processing, PPO and POD work in sequence to produce from catechins the theaflavins followed by further oxidation of the latter to thearubigins. These compounds are responsible for the color and taste/mouth-feel characteristics of tea. Limitation of molecular oxygen, degree of cell damage and length of the fermentation process can influence the final mix of the catechins, theaflavins and thearubigins. In the most limiting cases, white or green teas are produced with minimal transformation of catechins. More extensive or complete oxidation generates oolong and black teas, respectively, which are rich in theaflavins in the former, whilst in the latter almost complete conversion of catechins to theaflavins and thearubigins occurs.
- In vanilla beans, the phenolic precursors are mostly present as the β-D-glucosides. These glucosides are hydrolyzed enzymatically during the fermentation stage of curing to release the mono-phenols. These compounds are oxidized readily to dehydro-diphenols by POD/hydrogen peroxide. The other characterized dimeric phenylmethane compounds may also be generated by POD/PPO oxidation. PPO/molecular oxygen appears to be involved in oxidation of di-phenols, including chlorogenic and caffeic acid, especially to o-diphenols which can further react with amino acids or polymerize to form complex uncharacterized polyphenols. Opportunities exist within this latter oxidation process for the co-oxidation of mono-phenols. The vanilla phenol oxidation products comparable to the tea theaflavins/thearubigins are the dimeric diphenols and the diphenylmethanes. Some of these compounds contribute to the taste/mouth-feel of cured vanilla bean extracts. The brown coloration of cured vanilla beans is probably due to uncharacterized oxidation/polymerization of o-diphenols of the chlorogenic/caffeic family; a reaction initiated and dependent on the presence of molecular oxygen.
Tea Aroma
The volatile fractions of infusions of Chinese green cultivars, Longjing, Maofeng and Biluochun, produced by withering and pan-firing were analyzed by aroma extract dilution analysis [16]. A total of 58 odor active compounds with flavor dilution (FD) factors between 41 and 47 were identified. In all three cultivars and irrespective of the harvesting season, the following compounds—all with high FD factors—namely 4-hydroxy-2,5-dimethyl-3(2H)-furanone, 3-hydroxy-4,5-dimethyl-2(5H)-furanone, coumarin, vanillin, geraniol, (E)-isoeugenol and 2-methoxyphenol, were identified. These seven compounds are probably essential contributors to the aroma of Chinese green teas [17]. Of the above chemicals vanillin, geraniol and 2-methoxyphenol were probably present as glycosides in the green leaf and liberated during the withering and fermentation stages. The two furanones probably arise during the firing and drying stages of tea processing by Maillard reaction between the pentose sugars xylose, arabinose or ribose and the amino acids alanine or glycine [18].
Green tea
The floral compound (E)-isoeugenol was shown to originate from coniferyl alcohol, the latter being derived from the amino acid phenylalanine. The formation pathway for the phenylpropene (E)-isoeugenol in Petunia hybrida cv. Mitchell flowers were catalyzed by an enzyme that belongs to a structural family of NADPH dependent reductases. The petunia enzyme catalyzes the reduction of coniferyl acetate to (E)-isoeugenol by a direct reductive elimination or via a quinone methide intermediate [19, 20]. A petunia acetyltransferase was shown to be most active with coniferyl alcohol as the substrate. Suppression of the gene encoding the acetyltransferase resulted in the inhibition of (E)-isoeugenol biosynthesis suggesting this enzyme was the catalyst for conversion of coniferyl alcohol to the corresponding acetate; the latter being the substrate for the reductase conversion to (E)-isoeugenol [21]. (E)-isoeugenol may also arise by thermal degradation of coniferyl alcohol. Pyrolysis of a number of phenylpropanoids exhibited homolytic cleavage around C-O- and -O-H bonds. Pyrolysis of coniferyl alcohol, at 600oC, yielded 4-vinylguaiacol and (E)-isoeugenol as the major products with lesser amounts of vanillin, guaiacol and coniferyl aldehyde. The formation of (E)-isoeugenol was initiated by the elimination of the Cγ-hydroxyl group of coniferyl alcohol followed by addition of a proton to the resulting methylene radical. Further isomerization of (E)-isoeugenol to the (Z)- isomer can also occur but to a lesser extent [22].
Darjeeling Black Tea
The key aroma compounds of Darjeeling black tea were characterized by analysis of the volatile fraction from the tea infusion. Aroma extract dilution analysis of the 24 odor-active compounds with FD factors from 4-128, highlighted vanillin (vanilla-like), 4-hydroxy-2,5-dimethyl-3(2H)-furanone (caramel), 2-phenylethanol (flowery) and (E, E, Z)-2,4,6-nonatrienal (oat-flake-like) as the most odor potent compounds. Determined Odour Activity Values (OAV’s) highlighted (E ,E, Z)-nonatrienal, geraniol and linalool as key odorants in the infusion [23].
Black tea
As in the green tea volatiles above, the two terpene alcohols, geraniol and linalool, and the substituted phenol vanillin may arise by hydrolysis of their glycosidic precursors in the intact, young, leaf. Phenylethanol is formed in tomato fruit by the decarboxylation of phenylalanine to phenylamine. Removal of the –NH2 group generates phenylacetaldehyde which on reduction by an aldehyde reductase/NADPH realizes the aromatic alcohol [24]. Phenylethanol may also be present in the intact leaf as the glucoside. The trienoic compound (E, E, Z)-nonatrienal, first described in oat flake, is probably derived from linolenic acid by enzymatic or chemical oxidative routes [25].Transformation of amino acids by Strecker degradation to produce Cn-1 aldehydes catalyzed by the naturally occurring 1.2- or 1,4-quinones in tea dhool, cut/damaged tea leaf, at ambient temperatures occurs during fermentation [26]. This same reaction is also possible at elevated temperatures during the firing and drying process.
Carotenoids are precursors of aroma compounds particularly in oolong and black tea production via two main pathways. The enzymatic oxidation pathway is catalyzed by dioxygenases during fermentation which lead to oxy-scission products which by further transformations realize the final aroma compounds [27]. The most active precursor carotenoid are β-carotene, zeaxanthin and lutein. Oxidized tea flavanols-quinones formed during fermentation can also co-oxidize carotenoids to aroma active molecules including β-ionone, β-damascenone and theaspirone 28.
Cured Vanilla Aroma
At least 200 aroma compounds have been identified in solvent extracts of cured Vanilla planifolia beans by a combination of gas chromatography/ mass spectrometry (GC-MS) and high pressure liquid chromatography [29]. If we assume that this list encompasses all the molecules that are key for vanilla aroma, then simplification of the list from >200 to a manageable group is feasible. This can be achieved by identification of the key compounds and their quantification by GC-MS in conjunction with determination of FD factors[16]. This exercise was conducted on extracts of MV red Madagascan V. planifolia whole cured beans. AEDA of the sample detected 15 active odor compounds with FD factors of >125. The seven most important of these compounds included vanillin, guaiacol, ethyl-(E)-cinnamate, 2- and 3-methylbutanoate, β-damascenone and p-cresol [30]. The major flavor contribution of all 15 compounds was confirmed by reconstitution experiments. The compounded flavor based on these compounds showed strong similarity to the original MV red whole bean extract based on sensory evaluation of seven key vanilla aroma attributes.
Earlier work by Perez-Silva et al identified a total of 65 aroma compounds in solvent extracts of cured Mexican V. planifolia beans [31]. Of the total some 25 were considered to be the compounds accounting for most of the aroma of the cured beans (T-1)
Reconstitution experiments based on these 25 compounds, at their measured concentrations in the cured beans were sensorially similar to a Mexican vanilla extract [32]. The biochemical and/or chemical origin of most of the above aroma compounds has not been established though it is clear that virtually all are released or generated during curing. Vanillin and most of the phenols are present in the green beans as the β-D-glucosides and are liberated by hydrolysis primarily during the fermentation stage of curing [33]. Further transformation of some of the liberated phenols may occur to realize additional functionalized phenols. Non-phenolics may arise from polyunsaturated fatty acids by β-oxidation and/or fatty acid synthesis and from amino acids by Strecker type degradation[34]. These reactions realize short chain compounds, mainly unsaturated aldehydes, that contribute to the overall final flavor impact of cured vanilla beans [33, 35, 36]. It is clear that there are similarities in the profiles and pathways of formation of aroma compounds in tea processing and vanilla curing. In the case of the vanilla bean, the primary reaction involves release of the free phenols by hydrolysis of their β-D-glucosides. Secondary reactions involving further transformation of the liberated mono-phenols may occur under mild drying conditions employed. In tea processing a number of the important aroma compounds appear to rise during firing/drying. These include the furanones and perhaps (E)-isoeugenol. Geraniol, linalool and phenylethanol may be present in the green tea leaf as the glycosides, yielding the free alcohols on hydrolysis. The major difference between the two raw materials in terms of flavor generation may be a reflection of more extensive thermal treatment during tea processing.
Tea processing as indicated above can be very mild, white tea through green, through oolong to very extensive black tea. An outline of the processing regimes for the four tea types alongside the vanilla curing operation is shown in F-6.
The principal differences between the four tea processes are:
- The degree of tissue damage. This defines the potential for extensive oxidation of the endogenous catechins.
- The length of the fermentation stage. This dictates the extent of oxidation of catechins to theaflavins and thearubigins resulting in green through black/brown product appearance as well as the generation of characteristic aroma compounds. In unfermented teas, green notes predominate whereas in fully fermented teas aroma notes associated with Strecker/Maiillard reactions as well as carotenoid scission products appear.
- The absence or length of the firing and drying stages. Firing and drying stages enhance Strecker/Mailard and carotenoid scission reactions.
Comparison of the vanilla curing operation with that of tea processing shows some similarities.
In vanilla curing and tea processing they similarly require:
- Good quality raw material. For tea, the new, young leaves; whilst for vanilla, the ripe pods are utilized
- A tissue wounding stage. In the case of tea leaves, the wounding is induced by partial dehydration and subsequent tissue disruption by withering and leaf bruising. For vanilla beans, the wounding is initiated by subjecting the beans, usually by hot water immersion, at 60o-65oC, for ca.3 minutes. In both instances, cellular integrity is compromised to variable extents with consequent activation of enzymes, primarily hydrolases and oxido-reductases.
- A critical fermentation stage where free or liberated phenols are partially or totally oxidized by PPO/molecular oxygen and POD/hydrogen peroxide result in complex polymeric “brown” pigments that have mouth-feel and taste characteristics. In addition, aroma compounds are generated from simple phenols and amino acids as well as by degradation of carotenoids.
- An enzyme inactivation stage (firing) and subsequent drying stage that finalizes the formation of the mix of aroma compounds in the final product. This thermal process is important in the tea firing/drying process. During vanilla curing lower temperatures are employed resulting in extensive moisture loss with concomitant enzyme inactivation but perhaps limited thermal activity.
From a fixed base of reactants and enzymes in young tea leaves, a range of processing treatments realizes a wide spectrum of products with differing appearance, taste/mouth-feel and aroma character. The resulting product types include white, green, oolong and black teas. These different treatments as indicated above include:
- The degree of tissue damage. This defines the potential for extensive oxidation of the endogenous catechins
- The length of the fermentation stage. This dictates the extent of oxidation of catechins to theaflavins and thearubigins resulting in green through black/brown product appearance; as well as the generation of characteristic aroma compounds. In unfermented teas, green notes predominate; whereas in fully fermented teas, aroma notes associated with Strecker/Maillard reactions, as well as carotenoid scission products appear
- The absence or length of the firing and drying stages. Firing and drying stages enhance Strecker/Maillard reactions and potentially other chemistries.
Opportunities in Vanilla Curing
Traditional vanilla curing in processing terms has evolved into a fairly standard operation, as exemplified by the Bourbon process, which is virtually and universally adopted [37]. Despite this process constancy, there is little control or management of the individual stages of the operation leading to variability in product quality that manifest in part as reduced levels of vanillin. Tea processing, on the other hand, provides a selection of tea product types reflecting the processing variable outlined above.
Opportunities for novelty in vanilla curing can be evaluated with processing regimes based on tea treatments as follows:
- Limited damage to the ripe vanilla bean followed by drying to remove moisture. Color would be green and with aroma characteristic of the ripe bean and processing is equivalent to that of white tea.
- Limited damage to the ripe vanilla bean followed by heating/drying to give the equivalent of green tea. Color would be expected to be green to slight brown with aroma characteristic of the green bean with perhaps some phenolic notes.
- Extensive pod bruising or cutting followed by a short fermentation process. This stage is then followed by a heating/drying operation. This would be equivalent to oolong tea production. Color would be brown with aroma characterized by phenolic notes with perhaps some indication of caramellic notes.
- Extensive maceration of the whole vanilla bean followed by a long fermentation stage. To finish the process, elevated and extended heating would be invoked. The product would be expected to be dark brown in color with extensive conversion of phenolic glucosides to free phenols, as a consequence of extensive tissue damage, with characteristic phenolic notes and associated caramellic and other notes due to Strecker/Maillard type reaction products, e.g. isobutanal and 2-methylbutanal, and other thermal process notes [32].
Summary
Comparison of the phenolic composition between vanilla beans and tea leaves show similarities in terms of the mono-phenols, as the glucosides and the catechins, respectively. Both fresh tissues have active PPO/molecular oxygen and POD/hydrogen peroxide oxido-reductase activities. PPO seems to be associated principally with oxidation of ortho-di-phenols to the reactive o-quinones. These latter can undergo reaction with amino acids to form brown addition compounds or polymerize to form complex colored polyphenols. Though not characterized in taste terms, these compounds contribute at least to the color of the cured vanilla bean. In tea, the theaflavins and thearubigins are mostly responsible for tea color and taste/mouth-feel. Molecular oxygen is required for such reactions to occur. The o-quinones can additionally co-oxidise mono-phenols, such as vanillin or guaiacol, to carbon linked dimer and trimers with known taste/mouth-feel character. In addition, POD can oxidize mono-phenols to C-C linked di-phenols.
The processing of vanilla beans is a fairly unchanged series of operations which realize a general uniformity of flavor composition with some variability due to laxity in process control. Tea processing exhibits a flexibility resulting in virtually unprocessed white tea through to extensively treated black tea. Key features of this range of options include the extent of cellular damage, the absence or extended fermentation stage and finally the degree of heating and its intensity. Opportunities exist to model some vanilla curing operations on the spectrum of interventions, which represent tea processing. The ingenuity of vanilla curer can realize new directions for vanilla flavor based on exploitation of tea processing.
Acknowledgements
This paper recognizes the efforts of all the scientists and technologists whose knowledge and enthusiasm have brought us to our current understanding of the complexities of tea and vanilla chemistry, biochemistry and processing.